You’re watching Chennai Super Kings play Mumbai Indians at the Wankhede. The stands are packed and the atmosphere is electric. In the excitement, a bunch of people in one of the stands starts a Mexican wave. Everyone is eager to participate. At just the right moment, hundreds of people stand up and sit down in unison, giving the wave a full and fervent expression.
The Mexican wave is a type of wave that appears to propagate through a medium when the medium’s constituents are sequentially displaced from and restored to their original positions.
In the same way, let’s imagine a spin current.
A flow of spin
Every electron particle has an intrinsic property called quantum spin, or just spin for short. It represents some angular momentum carried by the particle. At any given moment it can be measured to have one of two values, colloquially called up and down. A spin current flows through a medium when electrons transmit a spin state — say, up — in quick succession before reverting to their original state (in this case down).
That is, when a spin current moves through a material, it implies a Mexican wave of a particular spin state moving through the material. The electrons themselves aren’t displaced, however.
Scientists are interested in spin currents because the electrons’ up and down spin states can represent 0s and 1s, to store and retrieve data in a computer’s hard drive. Spintronic drives of the future are expected to be able to store more data than existing drives, manipulate the data faster, and consume less energy.
In a study published in the journal Physical Review Letters on September 6, an international team of researchers reported an important advance on this front.
‘Crucial figure of merit’
Spintronics is a branch of physics dealing with the study and manipulation of electrons’ magnetic properties. Magnetic hard drives in computers already take advantage of spintronics. Each drive consists of a very thin magnetic disk that uses an effect called giant magnetoresistance to quickly store and read data encoded in the ups and downs of its electrons.
These spin states are modified by applying a magnetic field over small parts of the disc. The stronger the field, the faster the states change, and the faster the drive’s read/write speed. The field strength increased through the 1990s and 2000s as computers became a common sight in daily life. Today, drive speeds have been maxed out: new products improve the read/write speed only marginally.
Spin currents are expected to provide the next quantum leap on this frontier. If scientists can find a way to create spin currents rapidly, the currents can quickly reorganise electrons’ spin states and form the basis of next-generation drives. The catch is we need to produce spin currents that start-stop on an extremely short timescale. As a review article published in 2020 in the Journal of Magnetism and Magnetic Materials put it, “In spintronics, the spin-current generation efficiency is the most critical figure of merit for device applications.”
Beyond 1,000x better
Researchers are currently exploring ways to produce spin currents directly. In one existing scheme, they fire lasers at a material, delivering energy to its electrons and getting them to move around. Then they apply a magnetic field across the material so that some electrons’ spins are parallel to the field and some other electrons’ spins are anti-parallel. Finally they have the electrons interact with impurities in the material that scatter electrons with up and down spins at different rates, eventually leaving only electrons of one spin state behind.
A 2014 study by researchers at Eindhoven University of Technology in the Netherlands introduced another scheme. They sandwiched three layers of carefully chosen materials together. When they magnetised the uppermost layer and shot an ultrafast laser at the bottommost layer, the magnetisation of the middle layer changed in a way that induced spin currents in the uppermost layer.
These and other schemes have produced spin currents in the order of a few hundred femtoseconds (1 fs = 10-15 s). The lead author of the 2014 study, Sjors Schellekens, said in a press release that this timescale was “a factor 1,000” improvement on other technologies of the day. Schellekens also said the team was able to explain how the spin currents arose in the material, which is important to ensure the technique is reliable and there’s nothing in the data that simply resembles a spin current.
Soon, scientists began to anticipate even faster technologies.
For example, in January 2020, Science Advances published a paper by researchers from Germany, Sweden, and the U.S. They reported using a Heusler alloy to demonstrate the transfer of spin from one atom to another. Heusler alloys are compounds of some elements and which exhibit many properties of interest in spintronics. They anticipated their findings paved the way “towards spintronic devices that can operate on few-femtosecond or faster time scales.”
‘Petahertz clock rates’
In the September 6 study, researchers were able to use a new concept to produce spin currents in 2 fs.
Physicists with the Max Planck Institute for Microstructure Physics, Germany, had described this concept in a 2018 paper in Nano Letters and further fleshed it out in subsequent work. It was based on a mechanism called optical intersite spin transfer (OISTR). Here, light of specific frequencies could rapidly manipulate electrons’ angular momentum in a material without relying on indirect effects.
If it seems straightforward, deducing this mechanism wasn’t simple. The reason is light itself. In its wave form, it consists of an electric field and a magnetic field oscillating perpendicular to each other. When an electromagnetic wave interacts with matter, the material’s electronic properties respond almost immediately to the oscillating electric field. Translating the energy in the wave to the electrons’ spin is more long-winded, however, because it is mediated by intervening processes.
For some time, scientists were looking for evidence of a link between changes in the electrons’ spin with an incident light wave. In a 2019 study in Nature, researchers — including many involved in the 2018 study — found one way. They proposed using a layered stack of ferromagnetic materials like cobalt and nickel. Here, they wrote, “optical excitations result in the local displacement of charge carriers between different atomic species or across layer interfaces”. The result is OISTR, as a “spatially dislodged electron wave carries its spin away” from its ‘resident’ atom to another atom nearby.
Importantly, they were able to track subatomic changes in the stack using a bespoke “detection scheme” at the level of thousandths of femtoseconds. Their findings, they wrote, “paves the way towards coherent spintronic applications with petahertz clock rates”.
One-two punch
The researchers in the September 6 study engineered a material consisting of 20 alternating layers of cobalt and platinum. Each layer was less than 1 nm thick. They wrote, “This type of structure is ubiquitous in spintronic research, in particular to obtain giant magnetoresistance effects.” For their study, they added, the layered stack offered two advantages: it maximises “magneto-optical effects with normally incident light” and “multiplies the number of Co/Pt interfaces, so that potential injection taking place there becomes more measurable”.
They applied a magnetic field perpendicular to the stack to force the electrons to settle into an ordered arrangement of spins.
First the researchers fired a pulse of linearly polarised light only 4 fs long into the material. (When the oscillation of the electric field in the light wave is confined to a fixed plane, the light is linearly polarised.) This shot riled up the electrons and sent their spin states into a tizzy.
Right after, they shot another pulse of circularly polarised light (when the electromagnetic field is rotating around the light’s direction of motion). The way this light was absorbed as it passed through the material told the researchers how ‘well’ the electrons’ spins were ordered following the first laser shot.
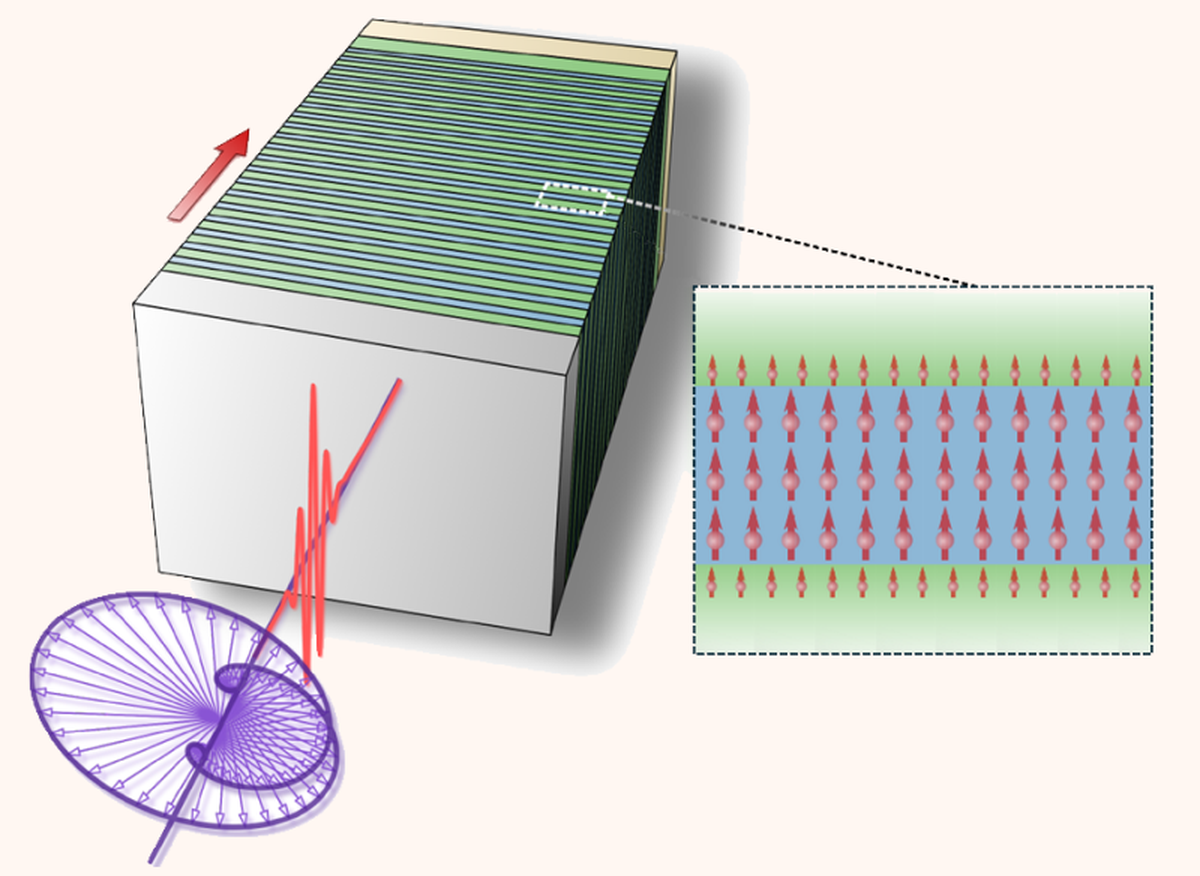
An illustration of the experimental setup in the new study. The red line shows the linearly polarised light, followed by the circularly polarised light in purple. The red arrow shows the direction of the applied magnetic field. Cobalt and platinum layers are shown in green and blue, respectively.
| Photo Credit:
Phys. Rev. Lett. 133, 106902
Proof of concept
The absorption of the circularly polarised light indicated to the researchers that in the cobalt layers, the electrons’ spins had become around 10% less ordered whereas they’d become slightly more ordered in the platinum layers — both within just 2 fs after the linearly polarised light had passed through.
The team developed a mathematical model to explain these findings using density-functional theory, which allows physicists to predict a material’s properties based on some fundamental quantum properties. These calculations are computationally intensive. The researchers made some assumptions to simplify their model, and this version showed that their findings could be explained if a small spin current had passed from the cobalt layers to the platinum layers in that 2-fs interval. Thus, the team reported a new record for the timescale at which a system could produce spin currents.
This is a proof of concept — a feat that says it’s possible for ultrafast lasers to directly induce spin currents within a few femtoseconds in a specific material. The researchers have said that next they plan to test whether femtosecond-laser pulses can produce useful spin currents in a functional spintronic device and whether they can produce spin currents in a few attoseconds, i.e. a thousand-times faster.
Published – September 13, 2024 09:19 am IST